|
Here are teaching slides for "Sea-glacier
dynamics and tropical sea-ice thickness". You can see a full-size version
by clicking on the thumbnail. You can then download that slide individually
by simply dragging it to your desktop.
|
|
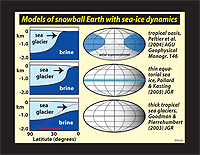 | 9.2:Three
models of snowball earth with sea-ice dynamics. Top: tropical oasis ("slushball")
model after Hyde et al. (2000) and Peltier et al. (2004). Middle: thin
equatorial marine ice model after McKay (2000) and Pollard & Kasting,
2005). Below: thick tropical sea glacier model ("hard snowball")
after Warren et al. (2002) and Goodman & Pierrehumbert (2003). The
three models require increasingly high levels of radiative forcing to deglaciate.
Differences between the models illustrate their sensitivity to parameters
like ice albedo in sublimation zones.
|
|
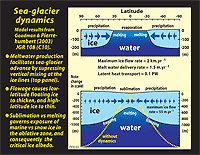 | 9.3:
Steady-state sea-glacier dynamics with ice lines close to the critical
latitude (top) and in snowball earth (bottom), based on the model results
of Goodman & Pierrehumbert (2003).
|
|
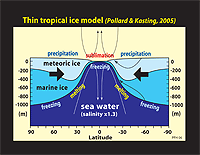 | 9.4:
Pole-to-pole cross-section of dynamic sea glaciers on an all-ocean snowball
planet according to the thin equatorial marine ice model of Pollard & Kasting
(2005). Equatorial ice is <2 m thick, allowing healthy rates of photosynthesis,
but the model is stable only when dark marine ice is exposed. If bubbly
meteoric ice encroaches into the equatorial zone, the ice rapidly thickens
due to higher albedo (see next slide).
|
|
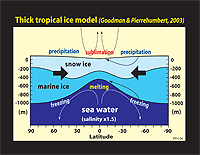 | 9.5:Pole-to-pole
cross-section of the dynamic sea glaciers on an all-ocean snowball planet
according to the thick tropical sea glacier model of Goodman & Pierrehumbert
(2003). Equatorward flow of sea glaciers is balanced by sublimation-condensation
and melting-freezing. Flowage causes thicker ice in the tropics and thinner
ice elsewhere, compared with static ice.
|
|
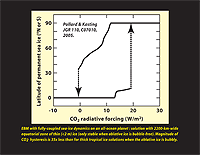 | 9.6:
Hysteresis in CO2-radiative forcing associated with a complete snowball "freeze-fry" cycle
assuming the thin equatorial marine ice solution of Pollard & Kasting
(2005). The amplitude in radiative forcing is 35x less than for the thick
tropical sea glacier model.
|
|
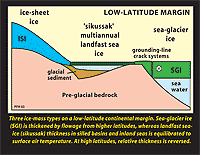 | 9.7:
Three hypothetical ice-mass types—ice-sheet ice, sea-glacier ice,
and multiannual landfast ice (sikkusak)—on a low-latitude continental
margin of a snowball earth (Halverson et al., 2005). Sikkusak thickness
is equilibrated to local surface air temperature (see slide 9-5) whereas
sea-glacier ice is thicker because of flowage from higher latitudes.
|
|
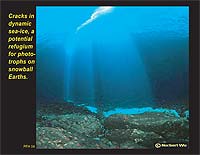 | 9.8:
Sea-glacier flowage means that crack systems will perpetually exist wherever
the moving ice impinges on the sea floor or landfast ice. Cracks propagate
through thick ice due to water pressure and freezing produces juvenile
(<5 years old) sea ice hosting brine channels populated by a variety
of prokaryotic and eukaryotic, phototrophic and heterotrophic organisms.
Because oxygen cannot diffuse from isolated brine channels, the organisms
must be tolerant of hyperoxia, a potentially useful pre-adaptation if atmospheric
oxygen levels rose following the younger Cryogenian snowball earth.
|
|
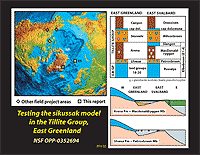 | 9.9:
Cryogenian and early Ediacaran successions in the East Greenland (EG)
and East Svalbard (ES) Caledonides. Glacial sedimentary interpretation
after Halverson et al. (2005).
|
|
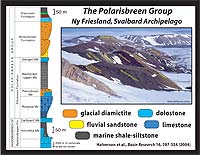 | 9.10:
Cryogenian and early Ediacaran succession in northeastern Svalbard. Note
the glacial marine Petrovbreen Member, the separate glacial terrestrial
Wilsonbreen Formation, and the MacDonaldryggen argillite and Slangen evaporitic
dolostone between the glacials. Conventional interpretion involves two
separate glaciations, possibly correlated with the older and younger Cryogenian
glaciations. Photo of section near Dracoisen, Ny Friesland, Spitsbergen.
|
|
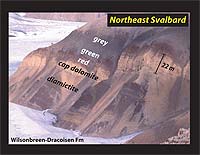 | 9.11:
Upper Wilsonbreen glacial diamictite and basal Dracoisen cap dolostone
in the
Ditlovtoppen section, Ny Friesland, Spitsbergen. The cap dolostone and
overlying strata are virtually indistinguishable from younger Cryogenian
(Marinoan) cap dolostones in East Greenland and NW Canada.
|
|
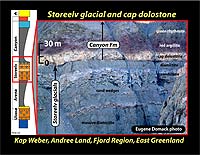 | 9.12:
Storeelv glacial diamictite and basal Canyon cap dolostone in the Andrée
Land section, Fjord Region, East Greenland.
|
|
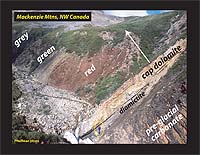 | 9.13:
Stelfox glacial diamictite and Ravensthroat cap dolostone in the Arctic
Red River section, Mackenzie Mountains, northern Canadian Cordillera.
|
|
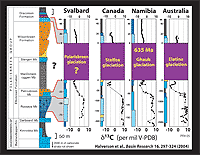 | 9.14:
Carbon isotopic records of the basal Dracoisen cap dolostone and the Russøya
Formation of the Polarisbreen Group, NE Svalbard, and apparent correlative
units in NW Canada, NW Namibia and South Australia. Correlation of the
Russøya and Trezona anomalies implies that both glacial horizons
(and inter-glacial strata) in Svalbard belong to the younger Cryogenian
(Marinoan) glaciation. Alternatively, a Trezona-like anomaly preceded the
older Cryogenian (Sturtian) glaciation in Svalbard.
|
|
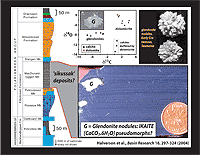 | 9.15:
Characteristic laminated argillite (suspension deposits) with early diagenetic
calcite nodules (glendonites, pseudomorphic after ikaaite) interpreted
as a sub-sikussak deposit sourced from basal ice-sheet meltwater plumes
(see slide 9-8). Ikaite is the preferred phase in cold phosphate-rich waters,
implying significant organic productivity possibly associated with crack
systems or clear marine ice <20 m thick.
|
|
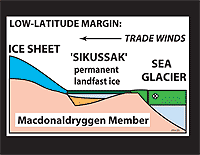 | 9.16:
Idealized three ice-mass types on a low-latitude continental margin on
a snowball earth. Paleomagnetic data (Maloof et al., in press, Bull. Geol.
Soc. Amer.) place East Svalbard in low southerly latitudes on the then
southest-facing (windward) margin of Laurentia.
|
|
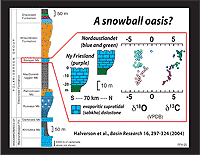 | 9.17:
Carbon and oxygen isotopic records for two sections of the Slangen Member,
an evaporitic carbonate parasequence between the two Polarisbreen glacials.
Contrasting δ13Ccarb records suggest diachronous
deposition associated with tidal flat progradation in an enclosed basin
with a rapidly changing
isotopic composition.
|
|
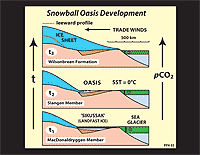 | 9.18:
Development of snowball “oases” (Halverson et al., 2005) in
areas of former sikkusak (low-latitude silled basins and inland seas).
Sikkusak disappears when sea-surface air temperatures reach the melting
point, while sea-glaciers continue their invasion of tropical ocean basins.
Opening of oases may alter vapor transport and hence the mass-balance of
adjacent ice sheets.
|
|
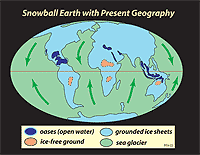 | 9.19:
Possible areas of oasis development on a hypothetical snowball earth with
present geography. Oases might occupy a few percent of global surface area.
Note sources of dust in low-lying ice-free terrestrial areas situated beneath
the descending arms of the atmospheric Hadley cells.
|
|
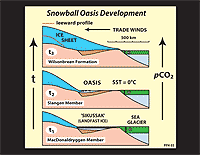 | 9.20:
Isotopic composition of δ13Ccarb in a snowball
oasis, compared with the present ocean. In the present ocean, depends on
the isotopic composition
of volcanic C input (~-5‰) and on steady-state organic burial forg.
Present atmospheric δ13C is equilibrated by the larger
oceanic C reservoir, and the equilibrium fractionation (at ~15ÅC) is ~-8‰.
On a snowball, atmospheric δ13C might be equilibrated
with seawater through air-sea gas exchange in cracks or with volcanic CO2 emissions,
which are ~-5‰,
or ~3‰ higher than the present atmosphere. In a snowball oasis, δ13Ccarb
will equilibrate to the larger atmospheric C reservoir. With surface temperature
~0ÅC, the equilibrium fractionation is ~10‰ (12‰ for CaCO3),
giving d13Ccarb as high as 7‰. As sikussak disappears, the isotopic
composition of oasis water might evolve rapidly if equilibration between
the atmosphere and ocean was not previously maintained through cracks.
|
|
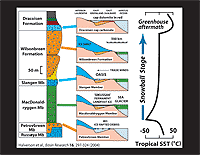 | 9.21:
Sikussak-oasis model for the Polarisbreen Group, in which the two glacial
horizons express
the onset and termination respectively of a single snowball epoch, the
Marinoan. The model is being tested by comparative studies in the more
landward correlative succession in East Greenland. This work is funded
through grants from the U.S. National Science Foundation, Arctic Natural
Science Division.
|